Research projects in the Storey lab address how neural progenitors arise and generate new nerve cells, and how they mature and respond to injury and cellular stress.
How do signals regulate neural differentiation onset?
The vertebrate nervous system first appears as a plate-like cell group in the early embryo. Most of the neural plate will form the brain, however a small cell group at one end form a stem zone, which progressively generates the spinal cord. Once cells leave the stem zone they begin to differentiate and so as development proceeds the temporal events of neural differentiation are spatially separated along the embryonic body. Current work in the lab addresses how changing signalling activity as cells leave the stem zone leads to the coordinated transcriptional onset of neural differentiation genes.
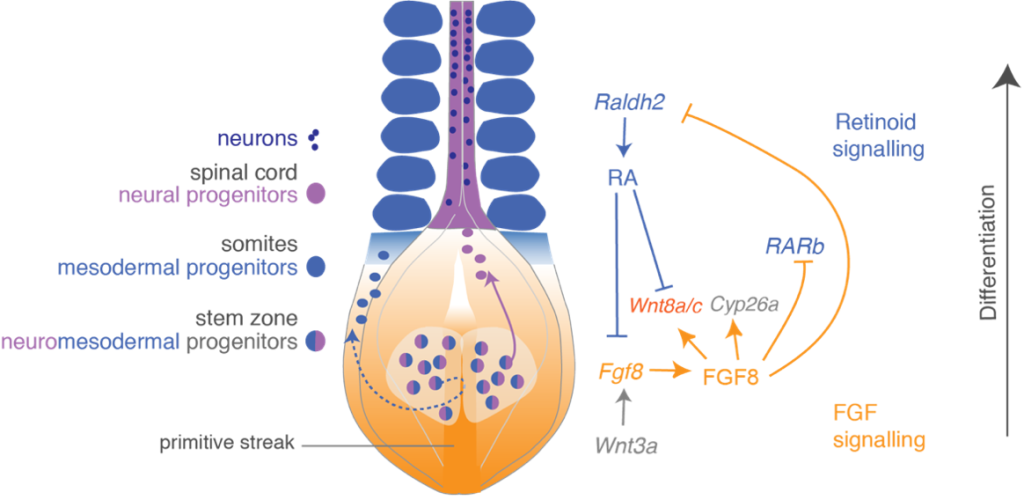
Our research has uncovered a signalling switch that controls the onset of neural differentiation. Cells in the stem zone (also known as the caudal lateral epiblast) express Fibroblast Growth Factors (FGFs) which keep these cells from differentiating, while Notch signalling helps to maintain them in the cell cycle. FGFs also maintain expression of a unique combination of stem zone genes, which include early neural and mesodermal genes, indeed cells co-expressing these genes are referred to as neuromesodermal progenitors. Cell labelling experiments have revealed that cells that will form mesoderm ingress through a unique tail-end structure called the primitive streak, while future neural cells remain in the epiblast layer, and, as they downregulate FGFs, go onto form the spinal cord. This transition is promoted by retinoic acid provided by adjacent mesoderm tissue, which both represses FGFs and promotes expression of neural differentiation and patterning genes. Conversely, FGF signalling inhibits synthesis of retinoic acid in the region of the stem zone and this mutual opposition of FGF and retinoid signalling thus controls and coordinates the onset of mesodermal and neural differentiation as the body elongates.
FGF signalling represses not only neuronal differentiation genes but also expression of ventral patterning genes. These have been shown by others to act in a combinatorial fashion to specify distinct types of neurons. A key regulator of ventral patterning is the signalling molecule sonic hedgehog, onset of transcription of which is also regulated by FGF signalling. So, opposition of FGF and retinoid signalling regulates start of neural differentiation and also specification of neuronal cell types. In further work we have identified roles for additional signalling pathways in this differentiation switch, including involvement of canonical Wnt signalling in regulation of the transition from FGF to retinoid signalling.
The FGF/retinoid signalling switch is conserved in other regions of the embryo, including the extending limb bud. Elevated FGF and impaired retinoid signalling are also characteristic of many cancers and this signalling switch therefore represents a fundamental mechanism that regulates cellular differentiation. Our recent work has shown that decline in the FGF signalling leads to increased chromatin accessibility at neural differentiation genes and implicates regulation of the polycomb repressive complex in this differentiation mechanism. Our current research using human pluripotent cells derived models for neural differentiation and phosopho-proteomic approaches, aims to uncover how decline in FGF-downstream ERK signalling regulates chromatin modifying proteins to coordinate expression of neural differentiation genes.
Selected publications
Semprich, C.I., Davidson, L., Amorim Torres, A., Patel H., Briscoe, J., Metzis, V., Storey, K.G. (2022). ERK1/2 signalling dynamics promote neural differentiation by regulating chromatin accessibility and the polycomb repressive complex PLoS Biology Dec 1;20(12):e3000221.doi: 10.1371/journal.pbio.3000221. PMID: 36455041
Verrier L, Davidson L, Gierliński M, Dady A, Storey KG. (2018) Neural differentiation, selection and transcriptomic profiling of human neuromesodermal progenitors-like cells in vitro. Development 145(16). pii: dev166215. doi: 10.1242/dev.166215. PMID: 29899136; PMC6124542
Patel, N.S., Rhinn, M., Semprich, C I., Halley, P.A., Dollé P., Bickmore, W.A., and Storey, K.G. (2013) FGF signalling regulates chromatin organisation during neural differentiation via mechanisms that can be uncoupled from transcription PLoS Genet. 2013, 9:e1003614.
Olivera-Martinez I, Harada H, Halley PA, Storey KG (2012) Loss of FGF-Dependent Mesoderm Identity and Rise of Endogenous Retinoid Signalling Determine Cessation of Body Axis Elongation. PLoS Biology 10(10): e1001415. doi:10.1371/journal.pbio.1001415
Stavridis, M. S., Lunn, J.S., Collins, B.J. and Storey K.G. (2007) A discrete period of FGF/Erk1/2 activity is required for vertebrate neural specification. Development 134, 2889-94.
Olivera-Martinez I.M., and Storey, K.G. (2007) Wnt signals provide a timing mechanism for the FGF/Retinoid differentiation switch in the extending body axis. Development 134, 2125-35.
Akai, J., Halley, P.A., and Storey, K.G. (2005) FGF dependent Notch signalling maintains the spinal cord stem zone. Genes & Development 19, 2877-87.
Delfino-Machín, M., Lunn, J.S., Breitkreuz, D.N., Akai, J. and Storey, K.G. (2005) Specification and maintenance of the spinal cord stem zone. Development 132, 4273-83
Diez del Corral, R., Olivera-Martinez, I., Goriely, A., Gale, E., Maden, M., and Storey, K (2003) Opposing FGF and Retinoid pathways control ventral neural patterning, neuronal differentiation and segmentation during body axis extension. Neuron 40, 65-79
Diez-del Corral, R. Breitkreuz, N. D., and Storey, K.G. (2002) Onset of neuronal differentiation in the spinal cord is regulated by the paraxial mesoderm and requires attenuation of FGF signalling. Development 129, 1681-91
Brown, J.M., and Storey, K.G. (2000). A region of the vertebrate neural plate in which neighbouring cells can adopt neural or epidermal cell fates. Current Biology 10, 869-872
Reviews
Henrique, D, Abranches, E., Verrier, L. and Storey, K.G. (2015) Neuromesodermal progenitors and the making of the spinal cord. Development 42(17):2864-75 (PMID: 26329597; PMCID: PMC4958456
Li, R.A., and Storey, K.G. (2011) An emerging molecular mechanism for the neural vs mesodermal cell fate decision. Cell Research, May;21(5):708-10. PMCID: PMC3203676
Wilson, V., Olivera-Martinez, I., and Storey, K.G. (2009) Stem cells, Signalling and Extension of the Vertebrate Body Axis Development 136, 1591-1604
How do cell biological mechanisms direct neuronal differentiation?
To understand better how signalling pathways influence cell behaviour within the developing neuroepithelium we established a live imaging assay that allows us to monitor individual cells within this tissue for several days. This revealed novel cell behaviours, including mis-localised and increased cell divisions in response to FGF signalling, and that alternation of division orientation can influence Notch signalling. This assay also allows us to investigate so called cell biological mechanisms that act inside neuroepithelial cells to mediate changes in cell shape and cell division and lead ultimately to the generation of new nerve cells.
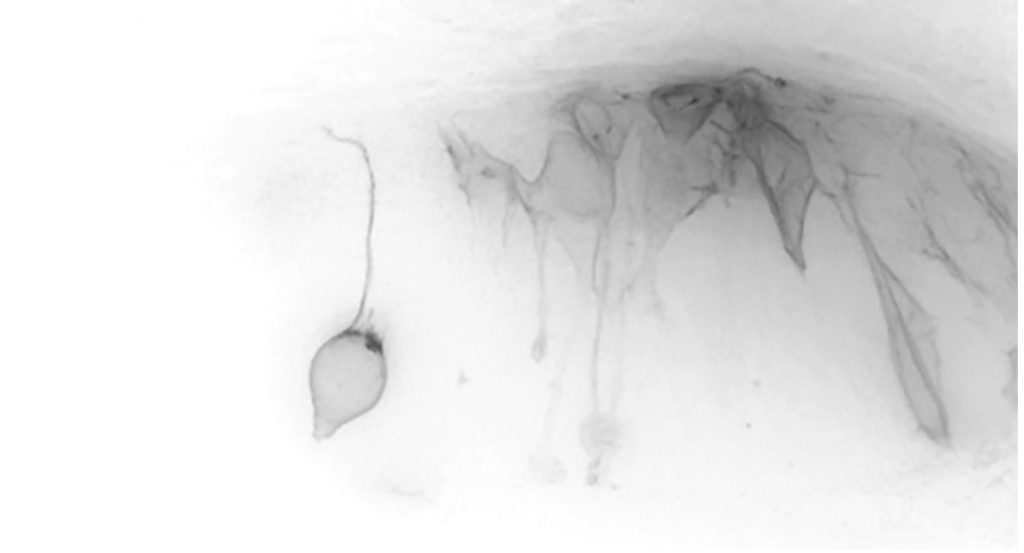
Movie still, neuroepithelial cell undergoing mitosis, while others
delaminate from the apical surface
The developing neuroepithelium is an ordered tissue, with cells extending across its width and their nuclei moving to the inner (apical) surface to undergo division and moving away (basally) as they enter G1 phase, and then either re-enter the cell cycle or exit to become a nerve cell. Using this live cell imaging approach, we have followed cells through the cell cycle and uncovered a new form of cell sub-division, apical abscission. This releases newborn nerve cells from the apical surface of the neuroepithelium, a process known as delamination. Apical abscission results in transient loss of apical cell polarity and disassembly of the key signalling organelle the primary cilium – steps which help to re-configure the cell for neuronal differentiation.

Apical tip of a newborn neuron undergoing apical abscission
Analysis of components of the cytoskeleton further revealed that neuronal delamination depends on interactions between actin filaments and microtubules and relies on the centrosome of the primary cilium, from which apical microtubules extend in a wheel-like configuration. Recent work investigates how cells interact within the neuroepithelium. This has uncovered a new form of cell-cell contact mediated by actin-dependent sub-apical lateral protrusions, which form a latticework between non-adjacent cells. Withdrawal lateral protrusions prefigures neuronal delamination raising the possibility that the lateral protrusions serve signalling function(s) which may regulate and pattern differentiation across the tissue.
Selected publications
Kasioulis I., Dady, A., James, J., Prescott, A., Halley, P.A., and Storey K.G., A lateral protrusion latticework connects neuroepithelial cells and is regulated during neurogenesis. J Cell Sci. 2022 Feb 25: jcs.259897. doi: 10.1242/jcs.259897. PMID: 35217862
Kasioulis I., Das, R.M., and Storey, K.G. (2017) Inter-dependent apical microtubule and actin dynamics orchestrate centrosome retention and neuronal delamination. eLife 2017;6:e26215. PMID: 29058679; PMCID: PMC5653239
Das, R.M. and Storey, K.G. (2014) Apical abscission alters cell polarity and dismantles the primary cilium during neurogenesis. Science 343, 200-204. PMID: 24408437; PMCID: PMC4066580
Das, R.M. and Storey, K.G. (2012) Mitotic spindle orientation can direct cell fate and bias Notch activity in chick neural tube. EMBO Reports 13(5): 448-54
Das, R.M., Wilcock, A.C., Swedlow, J.R., and Storey, K.G (2012). High-resolution live imaging of cell behaviour in the developing neuroepithelium. J. Vis. Exp. (62). pii: 3920. PMID: 22525126; PMCID: PMC3466664; DOI: 10.3791/3920
Vilas-Boas, F., Fior, R., Swedlow, J.R., StoreyK.G. and Henrique, D. (2011) A novel reporter of notch signalling indicates regulated and random notch activation during vertebrate neurogenesis. BMC Biology, 9: 58.
Wilcock, A.C., Swedlow, J.R. and Storey, K.G. (2007) Mitotic spindle orientation distinguishes stem cell and terminal modes of neuron production in the early spinal cord. Development 134, 1943-54
Reviews
Kasioulis, I and Storey, K.G. (2018) The cell biology of neurogenesis in the chick embryo ISDB special issue Int J Dev Biol. 2018;62(1-2-3):167-175. doi: 10.1387/ijdb.170268ks. PMID:29616725.
Das, R.M. and Storey, K.G. (2014b) Apical abscission, a novel cell-biological mechanism regulating neurogenesis. Neurogenesis 1, e29555.
How do spinal cord progenitors mature and respond to injury in adults?
As the spinal cord develops some neural progenitor cells in the proliferative ventricular layer are set aside as ependymal cells. These cells maintain many embryonic characteristics, including expression of neural progenitor proteins such as SOX2, and their retention in the adult spinal cord identifies a potential source of new cells for spinal cord repair. Following SOX2 expression has allowed us to monitor attrition of spinal cord progenitors in the developing mouse embryo. In collaboration with others, we have found that this cell population is refined by regulation of cell delamination mechanisms similar to those that mediate neuronal delamination.
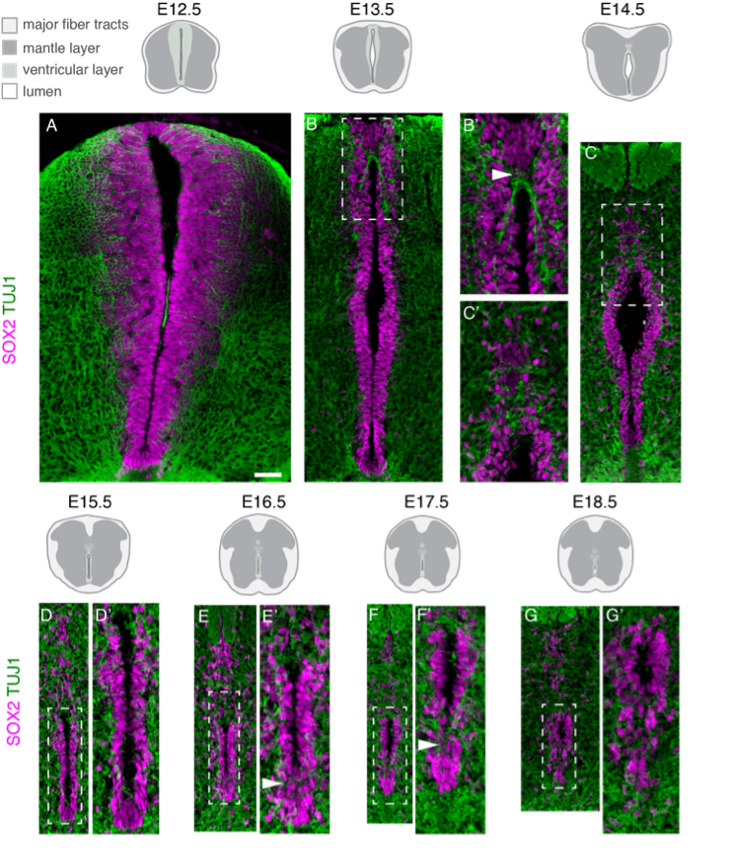
Attrition of neural progenitor cell population (pink) as the spinal cord develops
Current work focuses on characterising the adult spinal cord ependymal cell population: based on morphological features and the expression of a small number of molecular markers ependymal cells are rather heterogeneous. Moreover, it is unclear whether this reflects functional differences or distinct maturation states. To investigate this, we are using single-cell RNA-sequencing technologies to profile the transcriptomes of individual ependymal cells from the spinal cord of young adult and aged mice. To confirm computationally defined ependymal subtypes and states back in the tissue context, we use a combination of single-molecule fluorescence in situ hybridization (RNAscope) and immunofluorescence approaches. We also use explant cultures to model spinal cord injury and test novel hypothesis about how regenerative cell behaviour is regulated.
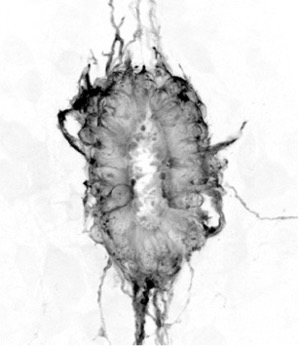
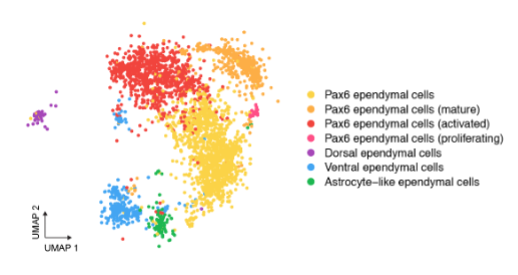
The adult spinal cord central canal is composed of heterogeneous ependymal cells; plot of single ependymal cells in the adult mouse spinal cord, each dot is a cell, colour-code based on computationally defined cell subtypes and states
Selected publications
Cañizares, M.A., Rodrigo Albors, A., Singer, G., Gorkic, M., Felts, P., Storey, K.G. (2020) Multiple steps mediate ventricular layer attrition to form the ependymal cell lining of the adult mouse spinal cord central canal. Journal of Anatomy, 236 (2), 334-350. PMID: 31670387, PMCID: PMC6956438
Tait CM, Chinnaiya K, Manning E, Murtaza M, Ashton JP, Furley N, Hill CJ, Alves CH, Wijnholds J, Erdmann KS, Furley A, Rashbass P, Das RM, Storey KG, Placzek M. Crumbs2 mediates ventricular layer remodelling to form the adult spinal cord central canal. PLoS Biol. 2020 Mar 9;18(3):e3000470. PMID:32150534; PMCID: PMC7108746
Rodrigo Albors, A., Singer G.A., May, A.P., Ponting, C.P. and Storey, K.G. (2023) An ependymal cell census identifies heterogeneous and ongoing cell maturation in the adult mouse spinal cord that changes dynamically on injury. Developmental Cell (in press).
How does cellular stress impact neural differentiation?
Environmental factors, such as dietary deficiencies, temperature extremes, viral infection and chemical pollutants are known to impact embryonic development. Human exposure to such factors is increasing and has acute and long-term health consequences. Despite detailed pathological descriptions of the phenotypic consequences of stressor exposure, little is known about the molecular and cellular response to stressors in the mammalian embryo. Moreover, the molecular pathways triggered by environmental stressors have been largely characterised in cell lines and their impact on developmental patterning and cell differentiation in vivo is poorly understood. Our recent analysis of embryos from a mouse mutant lacking the essential amino acid transporter (Slc7a5) revealed this to be a good model for investigating the impact of cellular stress in a physiologically relevant tissue context. Slc7a5 is broadly expressed in the embryo with higher levels in cell populations undergoing expansion or morphogenetic movements and Slc7a5-null embryos exhibit profound neural and limb bud outgrowth defects. Strikingly, they also show patterned induction of the Integrated Stress Response (ISR) in affected cell populations, revealing that some embryonic cell populations are particularly vulnerable to cellular stress. Current and future work includes establishment of in vitro models of human neuroepithelial differentiation to compare stress pathway activation and consequences between mouse and human tissues and will address whether the ISR normally plays a protective role and if it contributes to neural pathologies.
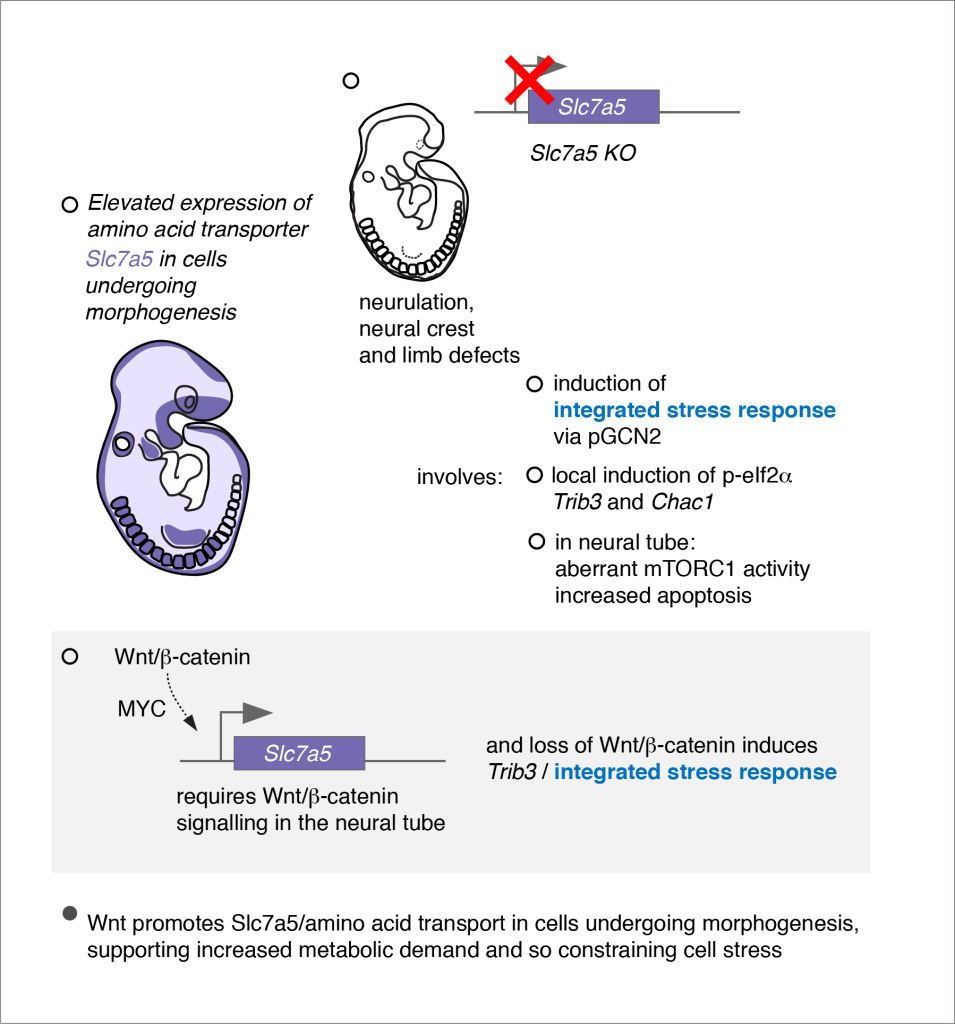
Selected publications
Poncet, N., Halley, P.A., Gierlińsk iM., Lipina C., Dady, A., Febrer M., Shi, Y-B., Yamaguchi, T.P., Taylor, P.M., Storey K.G. (2020) Wnt regulates amino acid transporter Slc7a5 and so constrains the integrated stress response in mouse embryos. EMBO Reports 21 (1), e48469. PMID: 31789450, PMCID: PMC6944906
Dady, A., Davidson, L., Halley, P.A, Storey, K.G. Human spinal cord differentiation proceeds rapidly in vitro and only initially maintains differentiation pace in a heterologous environment. Elife. 2022 Feb 21;11:e67283. doi: 10.7554/eLife.67283. PMID: 35188104 Read article